
Snake venom is a highly toxic saliva[1] containing zootoxins that facilitates in the immobilization and digestion of prey. This also provides defense against threats. Snake venom is injected by unique fangs during a bite, whereas some species are also able to spit venom.[2]
The glands that secrete zootoxins are a modification of the parotid salivary glands found in other vertebrates and are usually located on each side of the head, below and behind the eye, and enclosed in a muscular sheath. The venom is stored in large glands called alveoli before being conveyed by a duct to the base of channeled or tubular fangs through which it's ejected.[3][4]
Venom contains more than 20 different compounds, which are mostly proteins and polypeptides.[3][5] The complex mixture of proteins, enzymes, and various other substances has toxic and lethal properties.[2] Venom serves to immobilize prey.[6] Enzymes in venom play an important role in the digestion of prey,[4] and various other substances are responsible for important but non-lethal biological effects.[2] Some of the proteins in snake venom have very specific effects on various biological functions, including blood coagulation, blood pressure regulation, and transmission of nerve or muscle impulses. These venoms have been studied and developed for use as pharmacological or diagnostic tools, and even drugs.[2][5]
Chemistry
Proteins constitute 90-95% of venom's dry weight and are responsible for almost all of its biological effects.[5][7] The hundreds, even thousands, of proteins found in venom include toxins, neurotoxins in particular, as well as nontoxic proteins (which also have pharmacological properties), and many enzymes, especially hydrolytic ones.[2] Enzymes (molecular weight 13-150 KDa) make up 80-90% of viperid and 25-70% of elapid venoms, including digestive hydrolases, L-amino-acid oxidase, phospholipases, thrombin-like pro-coagulant, and kallikrein-like serine proteases and metalloproteinases (hemorrhagins), which damage vascular endothelium. Polypeptide toxins (molecular weight 5-10 KDa) include cytotoxins, cardiotoxins, and postsynaptic neurotoxins (such as α-bungarotoxin and α-Cobratoxin), which bind to acetylcholine receptors at neuromuscular junctions. Compounds with low molecular weight (up to 1.5 KDa) include metals, peptides, lipids, nucleosides, carbohydrates, amines, and oligopeptides, which inhibit angiotensin-converting enzyme (ACE) and potentiate bradykinin (BPP). Inter- and intra-species variation in venom chemical composition is geographical and ontogenic.[3] Phosphodiesterases interfere with the prey's cardiac system, mainly to lower the blood pressure. Phospholipase A2 causes hemolysis by lysing the phospholipid cell membranes of red blood cells.[8] Amino acid oxidases and proteases are used for digestion. Amino acid oxidase also triggers some other enzymes and is responsible for the yellow colour of the venom of some species. Hyaluronidase increases tissue permeability to accelerate the absorption of other enzymes into tissues. Some snake venoms carry fasciculins, like the mambas (Dendroaspis), which inhibit cholinesterase to make the prey lose muscle control.[9]
Type | Name | Origin |
---|---|---|
Oxidoreductases | lactate dehydrogenase | Elapidae |
L-amino-acid oxidase | All species | |
Catalase | All species | |
Transferases | Alanine amino transferase | |
Hydrolases | Phospholipase A2 | All species |
Lysophospholipase | Elapidae, Viperidae | |
Acetylcholinesterase | Elapidae | |
Alkaline phosphatase | Bothrops atrox | |
Acid phosphatase | Deinagkistrodon acutus | |
5'-nucleotidase | All species | |
Phosphodiesterase | All species | |
Deoxyribonuclease | All species | |
Ribonuclease 1 | All species | |
Adenosine triphosphatase | All species | |
Amylase | All species | |
Hyaluronidase | All species | |
NAD-Nucleotidase | All species | |
Kininogenase | Viperidae | |
Factor X activator | Viperidae, Crotalinae | |
Heparinase | Crotalinae | |
α-Fibrinogenase | Viperidae, Crotalinae | |
β-Fibrinogenase | Viperidae, Crotalinae | |
α-β-Fibrinogenase | Bitis gabonica | |
Fibrinolytic enzyme | Crotalinae | |
Prothrombin activator | Crotalinae | |
Collagenase | Viperidae | |
Elastase | Viperidae | |
Lyases | Glucosaminate ammonia-lyase |
Snake toxins vary greatly in their functions. The two broad classes of toxins found in snake venoms are neurotoxins (mostly found in elapids) and hemotoxins (mostly found in viperids). However, exceptions occur – the venom of the black-necked spitting cobra (Naja nigricollis), an elapid, consists mainly of cytotoxins, while that of the Mojave rattlesnake (Crotalus scutulatus), a viperid, is primarily neurotoxic. Both elapids and viperids may carry numerous other types of toxins.
α-neurotoxins | α-Bungarotoxin, α-toxin, erabutoxin, cobratoxin |
---|---|
β-neurotoxins (PLA2) | β-Bungarotoxin, Notexin, ammodytoxin, crotoxin, taipoxin |
κ-neurotoxins | Kappa-bungarotoxin |
Dendrotoxins (Kunitz) | Dendrotoxin, toxins I and K; possibly β-Bungarotoxin chain B |
Cardiotoxins | Naja nigricollis y-toxin, cardiotoxin III (aka cytotoxins) |
Myotoxins | Myotoxin-a, crotamine |
Sarafotoxins | Sarafotoxins a, b, and c |
Hemorrhagins (metalloprotease) | Mucrolysin, Atrolysins, Acutolysins, etc.[11] |
Hemotoxins (serine protease) | Venombin A |
Toxins
Neurotoxins
The beginning of a new neural impulse goes as follows:
- An exchange of ions (charged atoms) across the nerve cell membrane sends a depolarizing current towards the end of the nerve cell (cell terminus).
- When the depolarizing current arrives at the nerve cell terminus, the neurotransmitter acetylcholine (ACh), which is held in vesicles, is released into the space between the two nerves (synapse). It moves across the synapse to the postsynaptic receptors.
- ACh binds to the receptors and transfers the signal to the target cell, and after a short time, it's destroyed by acetylcholinesterase.
Fasciculins
- These toxins attack cholinergic neurons (those that use ACh as a transmitter) by destroying acetylcholinesterase (AChE). ACh, therefore, cannot be broken down and stays in the receptor. This causes tetany (involuntary muscle contraction), which can lead to death. The toxins have been called fasciculins since after injection into mice, they cause severe, generalized and long-lasting (5-7 h) fasciculations (rapid muscle contractions).
- Snake example: found mostly in the venom of mambas (Dendroaspis spp.) and some rattlesnakes (Crotalus spp.)
Dendrotoxins
- Dendrotoxins inhibit neurotransmissions by blocking the exchange of positive and negative ions across the neuronal membrane lead to no nerve impulse, thereby paralyzing the nerves.
- Snake example: mambas
α-neurotoxins
- Alpha-neurotoxins are a large group; over 100 postsynaptic neurotoxins having been identified and sequenced.[12] α-neurotoxins attack the Nicotinic acetylcholine receptors of cholinergic neurons. They mimic the shape of the acetylcholine molecule, and so fit into the receptors, where they block the ACh flow, leading to a feeling of numbness and paralysis.
- Snake examples: king cobra (Ophiophagus hannah) (known as hannahtoxin containing α-neurotoxins),[13] sea snakes (Hydrophiinae) (known as erabutoxin), many-banded krait (Bungarus multicinctus) (known as α-bungarotoxin), and cobras (Naja spp.) (known as cobratoxin)
Cytotoxins


Phospholipases
- Phospholipase is an enzyme that transforms the phospholipid molecule into a lysophospholipid (soap) → the new molecule attracts and binds fat and ruptures cell membranes. Phospholipase A2 is one specific type of phospholipases found in snake venom.
- Snake example: Okinawan habu (Trimeresurus flavoviridis)
Cardiotoxins / Cytotoxins
- Cardiotoxins are components that are specifically toxic to the heart. They bind to particular sites on the surface of muscle cells and cause depolarisation → the toxin prevents muscle contraction. These toxins may cause the heart to beat irregularly or stop beating, causing death. An example is the three-fingered cardiotoxin III from Chinese cobra, an example of the short three-fingered family (InterPro: IPR003572).
- Snake example: mambas, and some Naja species
Hemotoxins
- Hemotoxins cause hemolysis, the destruction of red blood cells (erythrocytes), or induce blood coagulation (clotting, e.g. mucrocetin). A common family of hemotoxins includes snake venom metalloproteinases such as mucrolysin.[11][14]
- Snake examples: most vipers and many cobra species: The tropical rattlesnake Crotalus durissus produces convulxin, a coagulant.[15]
Myotoxins
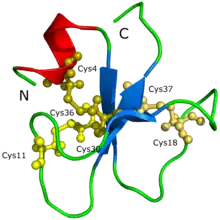
Myotoxins are small, basic peptides found in rattlesnake[16][17] and lizard (e.g. Mexican beaded lizard)[18] venoms. This involves a non-enzymatic mechanism that leads to severe skeletal muscle necrosis. These peptides act very quickly, causing instantaneous paralysis to prevent prey from escaping and eventually death due to diaphragmatic paralysis.
The first myotoxin to be identified and isolated was crotamine, discovered in the 1950s by Brazilian scientist José Moura Gonçalves from the venom of tropical South American rattlesnake Crotalus durissus terrificus. Its biological actions, molecular structure and gene responsible for its synthesis were all elucidated in the last two decades.
Determining venom toxicity (LD50)
Snake venom toxicity is assessed by a toxicological test called the median lethal dose, lethal dose 50% (abbreviated as LD50), which determines the concentration of a toxin required to kill half the members of a tested population. The potency of wild snake venom varies considerably because of assorted influences such as biophysical environment, physiological status, ecological variables, genetic variation (either adaptive or incidental), and other molecular and ecological evolutionary factors. This is true even for members of one species. Such variation is smaller in captive populations in laboratory settings, though it cannot be eliminated. However, studies to determine snake venom potency must be designed to minimize variability.
Several techniques have been designed to this end. One approach is to use 0.1% bovine serum albumin (also known as "fraction V" in Cohn process) as a diluent in determining LD50 values. It results in more accurate and consistent LD50 determinations than using 0.1% saline as a diluent. For example, fraction V produces about 95% purified albumin (dried crude venom). Saline as a diluent consistently produces widely varying LD50 results for nearly all venomous snakes. It produces unpredictable variation in precipitate purity (35-60%).[19] Fraction V is structurally stable because it has seventeen disulfide bonds; it's unique in that it has the highest solubility and lowest isoelectric point of major plasma proteins. This makes it the final fraction to be precipitated from its solution. Bovine serum albumin is located in fraction V. The precipitation of albumin is done by reducing the pH to 4.8, near the pH of the proteins, and maintaining the ethanol concentration at 40%, with a protein concentration of 1%. Thus, only 1% of the original plasma remains in the fifth fraction.[20]
When the ultimate goal of plasma processing is a purified plasma component for injection or transfusion, the plasma component must be highly pure. The first practical large-scale method of blood plasma fractionation was developed by Edwin J. Cohn during World War II. it's known as the Cohn process (or Cohn method). This process is also known as cold ethanol fractionation, as it involves gradually increasing the concentration of ethanol in the solution at 5 °C and 3 °C.[21] The Cohn Process exploits differences in plasma proteins properties, specifically, the high solubility and low pI of albumin. As the ethanol concentration is increased in stages from 0 to 40%, the pH declines from neutral (pH ~ 7) to about 4.8, which is near the pI of albumin.[21] At each stage, proteins are precipitated out of the solution and removed. The final precipitate is purified albumin. Several variations to this process exist, including an adapted method by Nitschmann and Kistler that uses fewer steps, and replaces centrifugation and bulk freezing with filtration and diafiltration.[21][22] Some newer methods of albumin purification add additional purification steps to the Cohn process and its variations. Chromatographic albumin processing emerged in the 1980s, however, it was not widely adopted until later due to the scarity of large-scale chromatography equipment. Methods incorporating chromatography generally begin with cryo-depleted plasma undergoing buffer exchange via either diafiltration or buffer exchange chromatography, to prepare the plasma for following ion exchange chromatography steps. After ion exchange, generally purification steps and buffer exchange occur.[21]
However, chromatographic methods began to be adopted in the 1980s. Developments were ongoing between when Cohn fractionation started emerge in 1946, and when chromatography emerged, in 1983. In 1962, the Kistler and Nistchmann process was created as a spin-off of the Cohn process. In the 1990s, the Zenalb and the CSL Albumex processes were created, which incorporated chromatography with variations. The general approach to using chromatography for plasma fractionation for albumin is: recovery of supernatant I, delipidation, anion exchange chromatography, cation exchange chromatography, and gel filtration chromatography. The recovered purified material is formulated with combinations of sodium octanoate and sodium N-acetyl tryptophanate and then subjected to viral inactivation procedures, including pasteurization at 60 °C. This is a more efficient alternative than the Cohn process because:
- smooth automation and a relatively inexpensive plant was needed,
- easier to sterilize equipment and maintain a good manufacturing environment
- chromatographic processes are less damaging to the albumin protein
- a more successful albumin result can be achieved.
Compared with the Cohn process, albumin purity increased from about 95% to 98% using chromatography, and the yield increased from about 65% to 85%. Small percentage increases make a difference in regard to sensitive measurements such as purity. The big drawback has to do with the economics. Although the method offered efficient, acquiring the necessary equipment was difficult. Large machinery is necessary, and for a long time, the lack of equipment availability limited its widespread use.
Evolution
Venom evolved just once among all Toxicofera about 170 million years ago, and then diversified into the huge venom diversity seen today.[23] The original toxicoferan venom was a very simple set of proteins that were assembled in a pair of glands. Subsequently, this set of proteins evolved independently in the various lineages of toxicoferans, including Serpentes, Anguimorpha, and Iguania.[24] Several snake lineages have since lost the ability to produce venom, often due to a change in diet or a change in predatory tactics.[23] In addition to this, venom strength and composition has changed due to changes in the prey of certain snake species. For example, the venom of the marbled sea snake (Aipysurus eydouxii) became significantly less toxic after the diet of this species changed from fish to strictly fish eggs.[23] The evolution of venom is thought to be responsible for the enormous expansion of snakes across the globe.[23][25]
The mechanism of evolution in most cases has been gene duplication in tissues unrelated to the venom.[24] Pre-existing salivary proteins are the likely ancestors of most venom toxin genes.[26] Expression of the new protein in the venom gland followed duplication.[24] Then proceeded natural selection for adaptive traits following the birth-and-death model, where duplication is followed by functional diversification, resulting in the creation of structurally related proteins that have slightly different functions.[23][24][27] The study of venom evolution has been a high priority for scientists in terms of scientific research, due to the medical relevance of snake venom, in terms of making antivenom and cancer research. Knowing more about the composition of venom and the ways it can potentially evolve is very beneficial. Three main factors that affect venom evolution have been closely studied: predators of the snake that are resistant to snake venom, prey that are in an evolutionary arms race with snakes, and the specific diets that affect the intraspecific evolution of venom.[23][28] Venoms continue to evolve as specific toxins and are modified to target a specific prey, and toxins are found to vary according to diet in some species.[29][30]
Rapid venom evolution can also be explained by the arms race between venom-targeted molecules in resistant predators, such as the opossum, and the snake venom that targets the molecules. Scientists performed experiments on the opossums and found that multiple trials showed replacement to silent substitutions in the von Willebrand factor (vWf) gene that encodes for a venom-targeted hemostatic blood protein. These substitutions are thought to weaken the connection between vWf and a toxic snake venom ligand (botrocetin), which changes the net charge and hydrophobicity. These results are significant to the venom evolution because it's the first citation of rapid evolution in a venom-targeted molecule. This shows that an evolutionary arms race may be occurring in terms of defensive purposes. Alternative hypotheses suggest that venom evolution is due to trophic adaption, whereas these scientists believe, in this case, that selection would occur on traits that help with prey survival in terms of venom evolution instead of predation success. Several other predators of the pit viper (mongooses and hedgehogs) show the same type of relationship between snakes, which helps to support the hypothesis that venom has a very strong defensive role along with a trophic role. Which in turn supports the idea that predation on the snakes can be the arms race that produces snake venom evolution.[31]
Some of the various adaptations produced by this process include venom more toxic to specific prey in several lineages,[30][32][33] proteins that pre-digest prey,[34] as well as a method to track down prey after a bite.[35] Though venom function has evolved to be specific to prey class (e.g. particular coagulatory effects),[36] the evolution of broad toxicological effects (e.g. neurotoxicity or coagulotoxicity) does not appear to be broadly affected by prey type.[37] The presence of digestive enzymes in snake venom was once believed to be an adaptation to assist digestion. However, studies of the western diamondback rattlesnake (Crotalus atrox), a snake with highly proteolytic venom, show that venom has no impact on the time required for food to pass through the gut.[38] These various adaptations of venom have also led to considerable debate about the definition of venom and venomous snakes.[23]
Injection
Vipers
In vipers, which have the most highly developed venom-delivery apparatus, the venom gland is very large and is surrounded by the masseter or temporal muscle, which consists of two bands, the superior arising from behind the eye, the inferior extending from the gland to the mandible. A duct carries venom from the gland to the fang. In vipers and elapids, this groove is completely closed, forming a hypodermic needle-like tube. In other species, the grooves are not covered, or only partially covered. From the anterior extremity of the gland, the duct passes below the eye and above the maxillary bone, to the basal orifice of the venom fang, which is ensheathed in a thick fold of mucous membrane. By means of the movable maxillary bone hinged to the prefrontal bone and connected with the transverse bone, which is pushed forward by muscles set in action by the opening of the mouth, the fang is erected and the venom discharged through the distal orifice. When the snake bites, the jaws close and the muscles surrounding the gland contract, causing venom to be ejected via the fangs.
Elapids
In the proteroglyphous elapids, the fangs are tubular, but are short and do not possess the mobility seen in vipers.
Colubrids
Opisthoglyphous colubrids have enlarged, grooved teeth situated at the posterior extremity of the maxilla, where a small posterior portion of the upper labial or salivary gland produces venom.
Mechanics of biting

Several genera, including Asian coral snakes (Calliophis), burrowing asps (Atractaspis), and night adders (Causus), are remarkable for having exceptionally long venom glands, extending along each side of the body, in some cases extending posterially as far as the heart. Instead of the muscles of the temporal region serving to press out the venom into the duct, this action is performed by those of the side of the body.
Considerable variability in biting behavior is seen among snakes. When biting, viperid snakes often strike quickly, discharging venom as the fangs penetrate the skin, and then immediately release. Alternatively, as in the case of a feeding response, some viperids (e.g. Lachesis) bite and hold. A proteroglyph or opisthoglyph may close its jaws and bite or chew firmly for a considerable time.
Differences in fang length between the various venomous snakes are likely due to the evolution of different striking strategies.[39] Additionally, it has been shown that the fangs of different species of venomous snakes have different sizes and shapes depending on the biomechanical properties of the snake's prey.[40]
Mechanics of spitting
Spitting cobras of the genera Naja and Hemachatus, when irritated or threatened, may eject streams or a spray of venom a distance of 4 to 8 ft. These snakes' fangs have been modified for the purposes of spitting; inside the fangs, the channel makes a 90° bend to the lower front of the fang. Spitters may spit repeatedly and still be able to deliver a fatal bite.
Spitting is a defensive reaction only. The snakes tend to aim for the eyes of a perceived threat. A direct hit can cause temporary shock and blindness through severe inflammation of the cornea and conjunctiva. Although usually no serious symptoms result if the venom is washed away immediately with plenty of water, blindness can become permanent if left untreated. Brief contact with the skin is not immediately dangerous, but open wounds may be vectors for envenomation.
Physiological effects
The four distinct types of venom act on the body differently:
- Proteolytic venom dismantles the molecular surroundings, including at the site of the bite.
- Hemotoxic venom acts on the cardiovascular system, including the heart and blood.
- Neurotoxic venom acts on the nervous system, including the brain.
- Cytotoxic venom has a localized action at the site of the bite.
Proteroglyphous snakes
The effect of the venom of proteroglyphous snakes (sea snakes, kraits, mambas, black snakes, tiger snakes, and death adders) is mainly on the nervous system, respiratory paralysis being quickly produced by bringing the venom into contact with the central nervous mechanism that controls respiration; the pain and local swelling that follow a bite are not usually severe. The bite of all the proteroglyphous elapids, even of the smallest and gentlest, such as the coral snakes, is, so far as known, deadly to humans. However, some mildly venomous elapids remain, such as the hooded snakes (Parasuta), bandy-bandies (Vermicella), etc.
Vipers
Viper venom (Russell's viper, saw-scaled vipers, bushmasters, and rattlesnakes) acts more on the vascular system, bringing about coagulation of the blood and clotting of the pulmonary arteries; its action on the nervous system is not great, no individual group of nerve-cells appears to be picked out, and the effect upon respiration is not so direct; the influence upon the circulation explains the great depression, which is a symptom of viperine envenomation. The pain of the wound is severe and is rapidly followed by swelling and discoloration. The symptoms produced by the bite of the European vipers are thus described by Martin and Lamb:[41]
The bite is immediately followed by the local pain of a burning character; the limb soon swells and becomes discolored, and within one to three hours great prostration, accompanied by vomiting, and often diarrhea, sets in. Cold, clammy perspiration is usual. The pulse becomes extremely feeble, and slight dyspnoea and restlessness may be seen. In severe cases, which occur mostly in children, the pulse may become imperceptible and the extremities cold; the patient may pass into coma. In from twelve to twenty-four hours these severe constitutional symptoms usually pass off; but in the meantime, the swelling and discoloration have spread enormously. The limb becomes phlegmonous and occasionally suppurates. Within a few days recovery usually occurs somewhat suddenly, but death may result from the severe depression or from the secondary effects of suppuration. That cases of death, in adults as well as in children, are not infrequent in some parts of the Continent is mentioned in the last chapter of this Introduction.
The Viperidae differ much among themselves in the toxicity of their venoms. Some, such as the Indian Russell's viper (Daboia russelli) and saw-scaled viper (E. carinatus); the American rattlesnakes (Crotalus spp.), bushmasters (Lachesis spp.), and lanceheads (Bothrops spp.); and the African adders (Bitis spp.), night adders (Causus spp.), and horned vipers (Cerastes spp.), cause fatal results unless a remedy is speedily applied. The bite of the larger European vipers may be very dangerous, and followed by fatal results, especially in children, at least in the hotter parts of the Continent; whilst the small meadow viper (Vipera ursinii), which hardly ever bites unless roughly handled, does not seem to be possessed of a very virulent venom, and although very common in some parts of Austria and Hungary, is not known to have ever caused a serious accident.
Opisthoglyphous colubrids
Biologists had long known that some snakes had rear fangs, 'inferior' venom injection mechanisms that might immobilize prey; although a few fatalities were on record, until 1957, the possibility that such snakes were deadly to humans seemed at most remote. The deaths of two prominent herpetologists, Robert Mertens and Karl Schmidt, from African colubrid bites, changed that assessment, and recent events reveal that several other species of rear-fanged snakes have venoms that are potentially lethal to large vertebrates.
Boomslang (Dispholidus typus) and twig snake (Thelotornis spp.) venoms are toxic to blood cells and thin the blood (hemotoxic, hemorrhagic). Early symptoms include headaches, nausea, diarrhea, lethargy, mental disorientation, bruising, and bleeding at the site and all body openings. Exsanguination is the main cause of death from such a bite.
The boomslang's venom is the most potent of all rear-fanged snakes in the world based on LD50. Although its venom may be more potent than some vipers and elapids, it causes fewer fatalities owing to various factors (for example, the fangs' effectiveness is not high compared with many other snakes, the venom dose delivered is low, and boomslangs are generally less aggressive in comparison to other venomous snakes such as cobras and mambas). Symptoms of a bite from these snakes include nausea and internal bleeding, and one could die from a brain hemorrhage and respiratory collapse.
Aglyphous snakes
Experiments made with the secretion of the parotid gland of Rhabdophis and Zamenis have shown that even aglyphous snakes are not entirely devoid of venom, and point to the conclusion that the physiological difference between so-called harmless and venomous snakes is only one of degree, just as various steps exist in the transformation of an ordinary parotid gland into a venom gland or of a solid tooth into a tubular or grooved fang.
Use of snake venoms to treat disease
Given that snake venom contains many biologically active ingredients, some may be useful to treat disease.[42]
For instance, phospholipases type A2 (PLA2s) from the Tunisian vipers Cerastes cerastes and Macrovipera lebetina have been found to have antitumor activity.[43] Anticancer activity has been also reported for other compounds in snake venom.[44][45] PLA2s hydrolyze phospholipids, thus could act on bacterial cell surfaces, providing novel antimicrobial (antibiotic) activities.[46]
The analgesic (pain-killing) activity of many snake venom proteins has been long known.[47][48] The main challenge, however, is how to deliver protein to the nerve cells: proteins usually are not applicable as pills.
Immunity
Among snakes
The question whether individual snakes are immune to their own venom has not yet been definitively settled, though an example is known of a cobra that self-envenomated, resulting in a large abscess requiring surgical intervention, but showing none of the other effects that would have proven rapidly lethal in prey species or humans.[49] Furthermore, certain harmless species, such as the North American common kingsnake (Lampropeltis getula) and the Central and South American mussurana (Clelia spp.), are proof against the venom of the crotalines, which frequent the same districts, and which they are able to overpower and feed upon. The chicken snake (Spilotes pullatus) is the enemy of the fer-de-lance (Bothrops caribbaeus) in St. Lucia, and in their encounters, the chicken snake is invariably the victor. Repeated experiments have shown the European grass snake (Natrix natrix) not to be affected by the bite of the European adder (Vipera berus) and the European asp (Vipera aspis), this being due to the presence, in the blood of the harmless snake, of toxic principles secreted by the parotid and labial glands, and analogous to those of the venom of these vipers. Several North American species of rat snakes, as well as king snakes, have proven to be immune or highly resistant to the venom of rattlesnake species. The king cobra, which does prey on cobras, is said to be immune to their venom.
Among other animals
The hedgehog (Erinaceidae), the mongoose (Herpestidae), the honey badger (Mellivora capensis) and the opossum are known to be immune to a dose of snake venom. Recently, the honey badger and domestic pig were found to have convergently evolved amino-acid replacements in their nicotinic acetylcholine receptor, which are known to confer resistance to alpha-neurotoxins in hedgehogs.[50] Whether the pig may be considered immune is still uncertain, though early studies show endogenous resistance in pigs tested against neurotoxins.[51] Though the pig's subcutaneous layer of fat may protect it against snake venom, most venoms pass easily through vascular fat layers, making this unlikely to contribute to its ability to resist venoms. The garden dormouse (Eliomys quercinus) has recently been added to the list of animals refractory to viper venom. Some populations of California ground squirrel (Otospermophilus beecheyi) are at least partially immune to rattlesnake venom as adults.
Among humans
The acquisition of human immunity against snake venom is ancient (from around 60 CE, Psylli tribe). Research into development of vaccines that will lead to immunity is ongoing. Bill Haast, owner and director of the Miami Serpentarium, injected himself with snake venom during most of his adult life, in an effort to build up an immunity to a broad array of venomous snakes, in a practice known as mithridatism. Haast lived to age 100, and survived a reported 172 snake bites. He donated his blood to be used in treating snake-bite patients when a suitable antivenom was not available. More than 20 so-treated individuals recovered.[52][53][54] Amateur researcher Tim Friede also lets venomous snakes bite him in the hopes of a vaccine against snake venom being developed, and has survived over 160 bites from different species as of January 2016.[55]
Traditional treatments
The World Health Organization estimates that 80% of the world's population depends on traditional medicine for their primary health-care needs.[56] Methods of traditional treatments of snakebites, although of questionable efficacy and perhaps even harmful, are nonetheless relevant.
Plants used to treat snakebites in Trinidad and Tobago are made into tinctures with alcohol or olive oil and kept in rum flasks called snake bottles, which contain several different plants and/or insects. The plants used include the vine called monkey ladder (Bauhinia cumanensis or Bauhinia excisa, Fabaceae), which is pounded and put on the bite. Alternatively, a tincture is made with a piece of the vine and kept in a snake bottle. Other plants used include mat root (Aristolochia rugosa), cat's claw (Pithecellobim unguis-cati), tobacco (Nicotiana tabacum), snake bush (Barleria lupulina), obie seed (Cola nitida), and wild gri gri root (Acrocomia aculeata). Some snake bottles also contain the caterpillars (Battus polydamas, Papilionidae) that eat tree leaves (Aristolochia trilobata). Emergency snake medicines are obtained by chewing a three-inch piece of the root of bois canôt (Cecropia peltata) and administering this chewed-root solution to the bitten subject (usually a hunting dog). This is a common native plant of Latin America and the Caribbean, which makes it appropriate as an emergency remedy. Another native plant used is mardi gras (Renealmia alpinia) (berries), which are crushed together with the juice of wild cane (Costus scaber) and given to the bitten. Quick fixes have included applying chewed tobacco from cigarettes, cigars, or pipes.[57] Making cuts around the puncture or sucking out the venom had been thought helpful in the past, but this course of treatment is now strongly discouraged, due to the risk of self-envenomation through knife cuts or cuts in the mouth (suction cups from snake bite kits can be used, but suctioning seldom provides any measurable benefit).[58][59]
Serotherapy
Serotherapy using antivenom is a common current treatment and has been described back in 1913.[note 1] Both adaptive immunity and serotherapy are specific to the type of snake; venom with identical physiological action do not cross-neutralize. Boulenger 1913 describes the following cases:
A European in Australia who had become immune to the venom of the deadly Australian tiger snake (Notechis scutatus), manipulating these snakes with impunity, and was under the impression that his immunity extended also to other species, when bitten by a lowland copperhead (Austrelaps superbus), an allied elapine, died the following day.
In India, the serum prepared with the venom of monocled cobra Naja kaouthia has been found to be without effect on the venom of two species of kraits (Bungarus), Russell's viper (Daboia russelli), saw-scaled viper (Echis carinatus), and Pope's pit viper (Trimeresurus popeiorum). Russell's viper serum is without effect on colubrine venoms, or those of Echis and Trimeresurus.
In Brazil, serum prepared with the venom of lanceheads (Bothrops spp.) is without action on rattlesnake (Crotalus spp.) venom.
Antivenom snakebite treatment must be matched as the type of envenomation that has occurred. In the Americas, polyvalent antivenoms are available that are effective against the bites of most pit vipers. Crofab is the antivenom developed to treat the bite of North American pit vipers.[60] These are not effective against coral snake envenomation, which requires a specific antivenom to their neurotoxic venom. The situation is even more complex in countries such as India, with its rich mix of vipers (Viperidae) and highly neurotoxic cobras and kraits of the Elapidae.
Notes
- ↑ This section is based on the 1913 book The Snakes of Europe, by G. A. Boulenger, which is now in the public domain in the United States (and possibly elsewhere) Because of its age, the text in this article should not necessarily be viewed as reflecting the current knowledge of snake venom
See also
References
- ↑ "Reptile Venom Research". Australian Reptile Park. Archived from the original on 2 February 2010. Retrieved 21 December 2010.
- 1 2 3 4 5 6 Bauchot R (1994). Snakes: A Natural History. New York City, NY, USA: Sterling Publishing Co., Inc. pp. 194–209. ISBN 978-1-4027-3181-5.
- 1 2 3 Halliday A, Kraig T, eds. (2002). Firefly Encyclopedia of Reptiles and Amphibians. Toronto, Canada: Firefly Books Ltd. pp. 202–203. ISBN 978-1-55297-613-5.
- 1 2 Bottrall JL, Madaras F, Biven CD, Venning MG, Mirtschin PJ (September 2010). "Proteolytic activity of Elapid and Viperid Snake venoms and its implication to digestion". Journal of Venom Research. 1 (3): 18–28. PMC 3086185. PMID 21544178.
- 1 2 3 4 Oliveira, Ana L.; Viegas, Matilde F.; da Silva, Saulo L.; Soares, Andreimar M.; Ramos, Maria J.; Fernandes, Pedro A. (July 2022). "The chemistry of snake venom and its medicinal potential". Nature Reviews Chemistry. 6 (7): 451–469. doi:10.1038/s41570-022-00393-7. ISSN 2397-3358. PMC 9185726. PMID 35702592.
- ↑ Mattison C (2007). The New Encyclopedia of Snakes. New Jersey, USA (first published in the UK): Princeton University Press (Princeton and Oxford) first published in Blandford. p. 117. ISBN 978-0-691-13295-2.
- ↑ Cardoso KC, Da Silva MJ, Costa GG, Torres TT, Del Bem LE, Vidal RO, et al. (October 2010). "A transcriptomic analysis of gene expression in the venom gland of the snake Bothrops alternatus (urutu)". BMC Genomics. 11 (1): 605. doi:10.1186/1471-2164-11-605. PMC 3017861. PMID 20977763.
- ↑ Condrea E, Devries A, Mager J (February 1964). "Hemolysis and splitting of human erythrocyte phospholipids by snake venoms". Biochimica et Biophysica Acta (BBA) - Specialized Section on Lipids and Related Subjects. 84 (1): 60–73. doi:10.1016/0926-6542(64)90101-5. PMID 14124757.
- ↑ Rodríguez-Ithurralde D, Silveira R, Barbeito L, Dajas F (1983). "Fasciculin, a powerful anticholinesterase polypeptide from Dendroaspis angusticeps venom". Neurochemistry International. 5 (3): 267–74. doi:10.1016/0197-0186(83)90028-1. PMID 20487949. S2CID 8952817.
- ↑ Mackessy, Stephen P. (1 May 2021). Mackessy, Stephen P. (ed.). Handbook of Venoms and Toxins of Reptiles (2 ed.). Second edition. | Boca Raton : CRC Press, 2021.: CRC Press. doi:10.1201/9780429054204. ISBN 978-0-429-05420-4.
{{cite book}}
: CS1 maint: location (link) - 1 2 "Keyword: Hemorrhagic toxin KW-1200". UniProt. Retrieved 1 June 2019.
- ↑ Hodgson WC, Wickramaratna JC (September 2002). "In vitro neuromuscular activity of snake venoms". Clinical and Experimental Pharmacology & Physiology. 29 (9): 807–14. doi:10.1046/j.1440-1681.2002.03740.x. PMID 12165047. S2CID 20158638.
- ↑ He YY, Lee WH, Zhang Y (September 2004). "Cloning and purification of alpha-neurotoxins from king cobra (Ophiophagus hannah)". Toxicon. 44 (3): 295–303. doi:10.1016/j.toxicon.2004.06.003. PMID 15302536.
- ↑ Bernardoni JL, Sousa LF, Wermelinger LS, Lopes AS, Prezoto BC, Serrano SM, Zingali RB, Moura-da-Silva AM (14 October 2014). "Functional variability of snake venom metalloproteinases: adaptive advantages in targeting different prey and implications for human envenomation". PLOS ONE. 9 (10): e109651. Bibcode:2014PLoSO...9j9651B. doi:10.1371/journal.pone.0109651. PMC 4196926. PMID 25313513.
- ↑ Hermans C, Wittevrongel C, Thys C, Smethurst PA, Van Geet C, Freson K (August 2009). "A compound heterozygous mutation in glycoprotein VI in a patient with a bleeding disorder". Journal of Thrombosis and Haemostasis. 7 (8): 1356–63. doi:10.1111/j.1538-7836.2009.03520.x. PMID 19552682. S2CID 205728095.
- ↑ Griffin PR, Aird SD (November 1990). "A new small myotoxin from the venom of the prairie rattlesnake (Crotalus viridis viridis)". FEBS Letters. 274 (1–2): 43–47. doi:10.1016/0014-5793(90)81325-I. PMID 2253781. S2CID 45019479.
- ↑ Samejima Y, Aoki Y, Mebs D (1991). "Amino acid sequence of a myotoxin from venom of the eastern diamondback rattlesnake (Crotalus adamanteus)". Toxicon. 29 (4–5): 461–468. doi:10.1016/0041-0101(91)90020-r. PMID 1862521.
- ↑ Whittington CM, Papenfuss AT, Bansal P, Torres AM, Wong ES, Deakin JE, et al. (June 2008). "Defensins and the convergent evolution of platypus and reptile venom genes". Genome Research. 18 (6): 986–994. doi:10.1101/gr.7149808. PMC 2413166. PMID 18463304.
- ↑ Broad AJ, Sutherland SK, Coulter AR (17 May 1979). "The lethality in mice of dangerous Australian and other snake venom" (PDF). Toxicon. 17 (6): 661–4. doi:10.1016/0041-0101(79)90245-9. PMID 524395.
- ↑ Rosen FS (31 July 2003). "Edwin J. Cohn and the Development of Protein Chemistry". The New England Journal of Medicine. 349 (5): 511–512. doi:10.1056/NEJM200307313490522.
- 1 2 3 4 Matejtschuk P, Dash CH, Gascoigne EW (December 2000). "Production of human albumin solution: a continually developing colloid". British Journal of Anaesthesia. 85 (6): 887–95. doi:10.1093/bja/85.6.887. PMID 11732525.
- ↑ Brodniewicz-Proba T (December 1991). "Human plasma fractionation and the impact of new technologies on the use and quality of plasma-derived products". Blood Reviews. 5 (4): 245–57. doi:10.1016/0268-960x(91)90016-6. PMID 1782484.
- 1 2 3 4 5 6 7 Fry BG, Casewell NR, Wüster W, Vidal N, Young B, Jackson TN (September 2012). "The structural and functional diversification of the Toxicofera reptile venom system". Toxicon. 60 (4): 434–48. doi:10.1016/j.toxicon.2012.02.013. PMID 22446061.
- 1 2 3 4 Casewell NR, Wüster W, Vonk FJ, Harrison RA, Fry BG (April 2013). "Complex cocktails: the evolutionary novelty of venoms". Trends in Ecology & Evolution. 28 (4): 219–29. doi:10.1016/j.tree.2012.10.020. PMID 23219381.
- ↑ Lomonte B, Fernández J, Sanz L, Angulo Y, Sasa M, Gutiérrez JM, Calvete JJ (June 2014). "Venomous snakes of Costa Rica: biological and medical implications of their venom proteomic profiles analyzed through the strategy of snake venomics". Journal of Proteomics. 105: 323–39. doi:10.1016/j.jprot.2014.02.020. PMID 24576642.
- ↑ Hargreaves, Adam D.; Swain, Martin T.; Hegarty, Matthew J.; Logan, Darren W.; Mulley, John F. (August 2014). "Restriction and Recruitment—Gene Duplication and the Origin and Evolution of Snake Venom Toxins". Genome Biology and Evolution. 6 (8): 2088–2095. doi:10.1093/gbe/evu166. ISSN 1759-6653. PMC 4231632. PMID 25079342.
- ↑ Lynch VJ (January 2007). "Inventing an arsenal: adaptive evolution and neofunctionalization of snake venom phospholipase A2 genes". BMC Evolutionary Biology. 7 (2): 2. doi:10.1186/1471-2148-7-2. PMC 1783844. PMID 17233905.
- ↑ Barlow A, Pook CE, Harrison RA, Wüster W (July 2009). "Coevolution of diet and prey-specific venom activity supports the role of selection in snake venom evolution". Proceedings. Biological Sciences. 276 (1666): 2443–2449. doi:10.1098/rspb.2009.0048. PMC 2690460. PMID 19364745.
- ↑ Pahari S, Bickford D, Fry BG, Kini RM (September 2007). "Expression pattern of three-finger toxin and phospholipase A2 genes in the venom glands of two sea snakes, Lapemis curtus and Acalyptophis peronii: comparison of evolution of these toxins in land snakes, sea kraits and sea snakes". BMC Evolutionary Biology. 7: 175. doi:10.1186/1471-2148-7-175. PMC 2174459. PMID 17900344.
- 1 2 Barlow A, Pook CE, Harrison RA, Wüster W (July 2009). "Coevolution of diet and prey-specific venom activity supports the role of selection in snake venom evolution". Proceedings: Biological Sciences. 276 (1666): 2443–9. doi:10.1098/rspb.2009.0048. JSTOR 30244073. PMC 2690460. PMID 19364745.
- ↑ Jansa SA, Voss RS (22 June 2011). "Adaptive evolution of the venom-targeted vWF protein in opossums that eat pitvipers". PLOS ONE. 6 (6): e20997. Bibcode:2011PLoSO...620997J. doi:10.1371/journal.pone.0020997. PMC 3120824. PMID 21731638.
- ↑ Calvete JJ, Ghezellou P, Paiva O, Matainaho T, Ghassempour A, Goudarzi H, Kraus F, Sanz L, Williams DJ (July 2012). "Snake venomics of two poorly known Hydrophiinae: Comparative proteomics of the venoms of terrestrial Toxicocalamus longissimus and marine Hydrophis cyanocinctus". Journal of Proteomics. 75 (13): 4091–101. doi:10.1016/j.jprot.2012.05.026. PMID 22643073.
- ↑ Li M, Fry BG, Kini RM (January 2005). "Eggs-only diet: its implications for the toxin profile changes and ecology of the marbled sea snake (Aipysurus eydouxii)". Journal of Molecular Evolution. 60 (1): 81–9. Bibcode:2005JMolE..60...81L. doi:10.1007/s00239-004-0138-0. PMID 15696370. S2CID 17572816.
- ↑ Mackessy SP (July 2010). "Evolutionary trends in venom composition in the western rattlesnakes (Crotalus viridis sensu lato): toxicity vs. tenderizers". Toxicon. 55 (8): 1463–74. doi:10.1016/j.toxicon.2010.02.028. PMID 20227433.
- ↑ Saviola AJ, Chiszar D, Busch C, Mackessy SP (March 2013). "Molecular basis for prey relocation in viperid snakes". BMC Biology. 11 (1): 20. doi:10.1186/1741-7007-11-20. PMC 3635877. PMID 23452837.
- ↑ Youngman, Nicholas J; Llinas, Joshua; Haworth, Mark; Gillett, Amber; Jones, Lee; Walker, Andrew A; Fry, Bryan G (June 2022). "Untangling interactions between Bitis vipers and their prey using coagulotoxicity against diverse vertebrate plasmas". Toxicon. 216: 37–44. doi:10.1016/j.toxicon.2022.06.012. ISSN 1879-3150. PMID 35780972. S2CID 250188352.
- ↑ Davies, Emma-Louise; Arbuckle, Kevin (December 2019). "Coevolution of Snake Venom Toxic Activities and Diet: Evidence that Ecological Generalism Favours Toxicological Diversity". Toxins. 11 (12): 711. doi:10.3390/toxins11120711. ISSN 2072-6651. PMC 6950196. PMID 31817769.
- ↑ McCue MD (October 2007). "Prey envenomation does not improve digestive performance in western diamondback rattlesnakes (Crotalus atrox)". Journal of Experimental Zoology Part A. 307 (10): 568–77. doi:10.1002/jez.411. PMID 17671964.
- ↑ Broeckhoven C, du Plessis A (August 2017). "Has snake fang evolution lost its bite? New insights from a structural mechanics viewpoint". Biology Letters. 13 (8): 20170293. doi:10.1098/rsbl.2017.0293. PMC 5582107. PMID 28768797.
- ↑ Cleuren SG, Hocking DP, Evans AR (June 2021). "Fang evolution in venomous snakes: Adaptation of 3D tooth shape to the biomechanical properties of their prey". Evolution; International Journal of Organic Evolution. 75 (6): 1377–1394. doi:10.1111/evo.14239. PMID 33904594. S2CID 233411378.
- ↑ Martin CJ, Lamb G (1907). "Snake-poison and Snake-bite". In Allbutt TC, Rolleston ND (eds.). A System of Medicine. London: MacMillan. pp. 783–821.
- ↑ McCleary RJ, Kini RM (February 2013). "Non-enzymatic proteins from snake venoms: a gold mine of pharmacological tools and drug leads". Toxicon. 62: 56–74. doi:10.1016/j.toxicon.2012.09.008. PMID 23058997.
- ↑ Zouari-Kessentini R, Srairi-Abid N, Bazaa A, El Ayeb M, Luis J, Marrakchi N (2013). "Antitumoral potential of Tunisian snake venoms secreted phospholipases A2". BioMed Research International. 2013: 1–9. doi:10.1155/2013/391389. PMC 3581298. PMID 23509718.
- ↑ Vyas VK, Brahmbhatt K, Bhatt H, Parmar U (February 2013). "Therapeutic potential of snake venom in cancer therapy: current perspectives". Asian Pacific Journal of Tropical Biomedicine. 3 (2): 156–62. doi:10.1016/S2221-1691(13)60042-8. PMC 3627178. PMID 23593597.
- ↑ Jain D, Kumar S (2012). "Snake venom: a potent anticancer agent". Asian Pacific Journal of Cancer Prevention. 13 (10): 4855–60. doi:10.7314/apjcp.2012.13.10.4855. PMID 23244070.
- ↑ de Oliveira Junior NG, e Silva Cardoso MH, Franco OL (December 2013). "Snake venoms: attractive antimicrobial proteinaceous compounds for therapeutic purposes". Cellular and Molecular Life Sciences. 70 (24): 4645–58. doi:10.1007/s00018-013-1345-x. PMID 23657358. S2CID 15127065.
- ↑ Woolf CJ (January 2013). "Pain: morphine, metabolites, mambas, and mutations". The Lancet. Neurology. 12 (1): 18–20. doi:10.1016/S1474-4422(12)70287-9. PMID 23237896. S2CID 8697382.
- ↑ Osipov A, Utkin Y (December 2012). "Effects of snake venom polypeptides on central nervous system". Central Nervous System Agents in Medicinal Chemistry. 12 (4): 315–28. doi:10.2174/187152412803760618. PMID 23270323. S2CID 36274766.
- ↑ "Sterile tail abscess in Naja annulifera - self-envenomation case". Archived from the original on 27 October 2004. Retrieved 2 April 2009.
- ↑ Drabeck D, Jansa S (2015). "Why the Honey Badger Doesn't Care: Independent Evolution of Resistance to Three Finger Toxins in the Nicotinic Acetylcholine Receptor". Toxicon. 99: 68–72. doi:10.1016/j.toxicon.2015.03.007. PMID 25796346.
- ↑ Grasset E, Zoutendykanda A, Schaafsma A (1935). "Studies on the toxic and antigenic properties of Southern African snake venoms with specialreference to the polyvalency of South African antivenene". Trans. R. Soc. Trop. Med. Hygiene. 28 (6): 601–612. doi:10.1016/S0035-9203(35)90031-1.
- ↑ "Farewell to these famous Floridians". Florida Trend. 19 December 2011. Retrieved 2 April 2012.
- ↑ Rosenberg C (21 June 2011). "Bill Haast dies at 100; snakes were the charm for south Florida celebrity". Los Angeles Times. Retrieved 16 October 2012.
- ↑ Schudel M (18 June 2011). "Bill Haast dies at 100: Florida snake man provided venom for snakebite serum". The Washington Post. Retrieved 16 October 2012.
- ↑ "Man makes deadly snakes bite him 160 times in hunt for human antidote | Americas | News | The Independent". Independent.co.uk. 21 January 2016. Retrieved 7 July 2016.
- ↑ Hiremath VT, Taranath TC (February 2010). "Traditional Phytotherapy for Snake bites by Tribes of Chitradurga District, Karnataka, India". Ethnobotanical Leaflets. 14 (2): 120–125.
- ↑ Zethelius M, Balick MJ (March 1982). "Modern medicine and shamanistic ritual: a case of positive synergistic response in the treatment of a snakebite" (PDF). Journal of Ethnopharmacology. 5 (2): 181–5. doi:10.1016/0378-8741(82)90042-3. PMID 7057657.
- ↑ "Treating Snake Bites". Ces.ncsu.edu. Retrieved 16 October 2012.
- ↑ "CDC - Venomous Snakes - NIOSH Workplace Safety and Health Topic". CDC.gov. 1 July 2016. Retrieved 7 July 2016.
- ↑ http://www.savagelabs.com/Products/CroFab/Home/crofab_frame.htm Archived 3 March 2016 at the Wayback Machine Link to PDF for full prescribing information, retrieved 11/12/12
Further reading
- Jonassen I, Collins JF, Higgins DG (August 1995). "Finding flexible patterns in unaligned protein sequences". Protein Science. 4 (8): 1587–95. doi:10.1002/pro.5560040817. PMC 2143188. PMID 8520485.
- Shaw IC (2007). "Chapter 19: Snake Toxins". In Waring RH, Steventon GB, Mitchell SC (eds.). Molecules of Death (Second ed.). River Edge, N.J: Imperial College Press. pp. 329–344. ISBN 978-1-86094-815-2.
External links

- An overview of the diversity and evolution of snake fangs.
- Snake VenomsUMich Orientation of Proteins in Membranes families/superfamily-55 - Calculated orientations of snake venom phospholipases A2 and myotoxins in the lipid bilayer.
- LD50's for most toxic venoms.
- Australian Venom Research Unit - a general source of information for venomous creatures in Australia.
- biomedcentral.com - Medicinal and ethnoveterinary remedies of hunters in Trinidad.
- reptilis.net - How venom works.
- snakevenom.net - Drying and storage of snake venom.